Science and policy characteristics of the Paris Agreement temperature goal
The Paris Agreement sets a long-term temperature goal of holding the global average temperature increase to well below 2 °C, and pursuing efforts to limit this to 1.5 °C above pre-industrial levels. Here, we present an overview of science and policy aspects related to this goal and analyse the implications for mitigation pathways. We show examples of discernible differences in impacts between 1.5 °C and 2 °C warming. At the same time, most available low emission scenarios at least temporarily exceed the 1.5 °C limit before 2100. The legacy of temperature overshoots and the feasibility of limiting warming to 1.5 °C, or below, thus become central elements of a post-Paris science agenda. The near-term mitigation targets set by countries for the 2020–2030 period are insufficient to secure the achievement of the temperature goal. An increase in mitigation ambition for this period will determine the Agreement's effectiveness in achieving its temperature goal.
This is a preview of subscription content, access via your institution
Access options
Subscribe to this journal
Receive 12 print issues and online access
206,07 € per year
only 17,17 € per issue
Buy this article
Prices may be subject to local taxes which are calculated during checkout
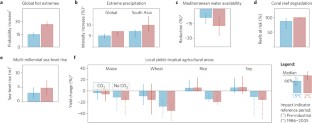

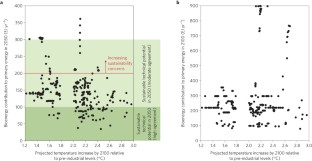
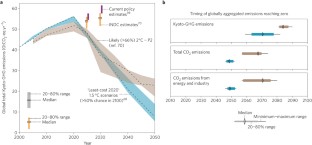
Similar content being viewed by others
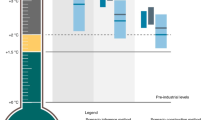
Wave of net zero emission targets opens window to meeting the Paris Agreement
Article 16 September 2021
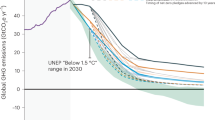
Ratcheting of climate pledges needed to limit peak global warming
Article 10 November 2022
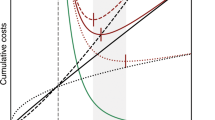
Paris Climate Agreement passes the cost-benefit test
Article Open access 27 January 2020
References
- United Nations Framework Convention on Climate Change (UNFCCC, 1992).
- Knutti, R., Rogelj, J., Sedláček, J. & Fischer, E. M. A scientific critique of the two-degree climate change target. Nature Geosci.9, 13–18 (2015). ArticleCASGoogle Scholar
- Adoption of the Paris Agreement FCCC/CP/2015/10/Add.1 (UNFCCC, 2015).
- Hare, W. L., Cramer, W., Schaeffer, M., Battaglini, A. & Jaeger, C. C. Climate hotspots: key vulnerable regions, climate change and limits to warming. Reg. Environ. Change11, 1–13 (2011). ArticleGoogle Scholar
- IPCC Climate Change 2001: Impacts, Adaptation, and Vulnerability (eds McCarthy, J. J., Canziani, O. F., Leary, N. A., Dokken, D. J. & White, K. S.) (Cambridge Univ. Press, 2001).
- Smith, J. B. et al. Assessing dangerous climate change through an update of the intergovernmental panel on climate change (IPCC) “reasons for concern”. Proc. Natl Acad. Sci. USA106, 4133–4137 (2009). ArticleCASGoogle Scholar
- Oppenheimer, M. et al. in Climate Change: Impacts, Adaptation, and Vulnerability (eds Field, C. B. et al.) 1039–1099 (IPCC, Cambridge Univ. Press, 2014). Google Scholar
- IPCC Climate Change 2007: Synthesis Report (eds Pachauri, R. K. & Reisinger, A.) (Cambridge Univ. Press, 2007).
- Submissions from Parties FCCC/KP/AWG/2009/MISC.1/Add.1 (UNFCCC, 2009).
- The Copenhagen Accord FCCC/CP/2009/11/Add.1 (UNFCCC, 2009).
- The Cancun Agreements FCCC/CP/2010/7/Add.1 (UNFCCC, 2010).
- Report on the Structured Expert Dialogue on the 2013–2015 Review FCCC/SB/2015/INF.1 (UNFCCC, 2015).
- Seneviratne, S. I., Donat, M. G., Pitman, A. J., Knutti, R. & Wilby, R. L. Allowable CO2 emissions based on regional and impact-related climate targets. Nature529, 477–483 (2016). ArticleCASGoogle Scholar
- IPCC Climate Change 2013: The Physical Science Basis (eds Stocker, T. F. et al.) (Cambridge Univ. Press, 2013).
- IPCC Managing the Risks of Extreme Events and Disasters to Advance Climate Change Adaptation (eds Field, C. B. et al.) (Cambridge Univ. Press, 2012).
- Seneviratne, S. I., Donat, M. G., Mueller, B. & Alexander, L. V. No pause in the increase of hot temperature extremes. Nature Clim. Change4, 161–163 (2014). ArticleGoogle Scholar
- Fischer, E. M. & Knutti, R. Anthropogenic contribution to global occurrence of heavy-precipitation and high-temperature extremes. Nature Clim. Change5, 560–564 (2015). ArticleGoogle Scholar
- Greve, P. et al. Global assessment of trends in wetting and drying over land. Nature Geosci.7, 716–721 (2014). ArticleCASGoogle Scholar
- Westra, S., Alexander, L. V. & Zwiers, F. W. Global increasing trends in annual maximum daily precipitation. J. Clim.26, 3904–3918 (2013). ArticleGoogle Scholar
- Lehmann, J., Coumou, D. & Frieler, K. Increased record-breaking precipitation events under global warming. Climatic Change132, 501–515 (2015). ArticleGoogle Scholar
- Schleussner, C.-F. et al. Differential climate impacts for policy relevant limits to global warming: the case of 1.5 °C and 2 °C. Earth Syst. Dynam.7, 327–351 (2016). ArticleGoogle Scholar
- Sedláček, J. & Knutti, R. Half of the world's population experience robust changes in the water cycle for a 2 °C warmer world. Environ. Res. Lett.9, 044008 (2014). ArticleGoogle Scholar
- Schewe, J. et al. Multimodel assessment of water scarcity under climate change. Proc. Natl Acad. Sci. USA111, 3245–3250 (2014). ArticleCASGoogle Scholar
- Rosenzweig, C. et al. Assessing agricultural risks of climate change in the 21st century in a global gridded crop model intercomparison. Proc. Natl Acad. Sci. USA111, 3268–3273 (2013). ArticleCASGoogle Scholar
- McGrath, J. M. & Lobell, D. B. Regional disparities in the CO2 fertilization effect and implications for crop yields. Environ. Res. Lett.8, 014054 (2013). ArticleCASGoogle Scholar
- Tai, A. P. K., Martin, M. V. & Heald, C. L. Threat to future global food security from climate change and ozone air pollution. Nature Clim. Change4, 817–821 (2014). ArticleCASGoogle Scholar
- Challinor, A. J. et al. A meta-analysis of crop yield under climate change and adaptation. Nature Clim. Change4, 287–291 (2014). ArticleGoogle Scholar
- Elliott, J. et al. Constraints and potentials of future irrigation water availability on agricultural production under climate change. Proc. Natl Acad. Sci. USA111, 3239–3244 (2013). ArticleCASGoogle Scholar
- Bodirsky, B. L. et al. Reactive nitrogen requirements to feed the world in 2050 and potential to mitigate nitrogen pollution. Nature Commun.5, 3858 (2014). ArticleCASGoogle Scholar
- Deryng, D., Conway, D., Ramankutty, N., Price, J. & Warren, R. Global crop yield response to extreme heat stress under multiple climate change futures. Environ. Res. Lett.9, 034011 (2014). ArticleGoogle Scholar
- Nelson, G. C. et al. Agriculture and climate change in global scenarios: why don't the models agree. Agric. Econ.45, 85–101 (2014). ArticleGoogle Scholar
- Lesk, C., Rowhani, P. & Ramankutty, N. Influence of extreme weather disasters on global crop production. Nature529, 84–87 (2016). ArticleCASGoogle Scholar
- Asseng, S. et al. Rising temperatures reduce global wheat production. Nature Clim. Change5, 143–147 (2015). ArticleGoogle Scholar
- Pörtner, H.-O. et al. in Climate Change 2014: Impacts, Adaptation, and Vulnerability (Field, C. B. et al.) Ch. 6 (IPCC, Cambridge Univ. Press, 2014). Google Scholar
- Gattuso, J.-P. et al. Contrasting futures for ocean and society from different anthropogenic CO2 emissions scenarios. Science349, aac4722 (2015). ArticleCASGoogle Scholar
- Meissner, K. J., Lippmann, T. & Sen Gupta, A. Large-scale stress factors affecting coral reefs: open ocean sea surface temperature and surface seawater aragonite saturation over the next 400 years. Coral Reefs31, 309–319 (2012). ArticleGoogle Scholar
- Frieler, K. et al. Limiting global warming to 2 °C is unlikely to save most coral reefs. Nature Clim. Change3, 165–170 (2013). ArticleGoogle Scholar
- Hezel, P. J., Fichefet, T. & Massonnet, F. Modeled Arctic sea ice evolution through 2300 in CMIP5 extended RCPs. Cryosphere8, 1195–1204 (2014). ArticleGoogle Scholar
- Burke, M., Hsiang, S. M. & Miguel, E. Global non-linear effect of temperature on economic production. Nature527, 235–239 (2015). ArticleCASGoogle Scholar
- Mathesius, S., Hofmann, M., Caldeira, K. & Schellnhuber, H. J. Long-term response of oceans to CO2 removal from the atmosphere. Nature Clim. Change5, 1107–1113 (2015). ArticleCASGoogle Scholar
- Schewe, J., Levermann, A. & Meinshausen, M. Climate change under a scenario near 1.5 °C of global warming: monsoon intensification, ocean warming and steric sea level rise. Earth Syst. Dynam.2, 25–35 (2011). ArticleGoogle Scholar
- Clark, P. U. et al. Consequences of twenty-first-century policy for multi-millennial climate and sea-level change. Nature Clim. Change6, 360–369 (2016). ArticleGoogle Scholar
- Schneider von Deimling, T. et al. Estimating the near-surface permafrost-carbon feedback on global warming. Biogeosci.9, 649–665 (2012). ArticleCASGoogle Scholar
- Levermann, A. et al. The multimillennial sea-level commitment of global warming. Proc. Natl Acad. Sci. USA110, 13745–13750 (2013). ArticleCASGoogle Scholar
- Dutton, A. et al. Sea-level rise due to polar ice-sheet mass loss during past warm periods. Science349, aaa4019 (2015). ArticleCASGoogle Scholar
- Mace, M. J. Mitigation commitments under the Paris Agreement and the way forward. Clim. Law6, 21–39 (2016). ArticleGoogle Scholar
- Decision IPCC/XLIII-7 (IPCC, 2016).
- Clarke, L. et al. in Climate Change 2014: Mitigation of Climate Change (Edenhofer, O. et al.) Ch. 6 (IPCC, Cambridge Univ. Press, 2014). Google Scholar
- IPCC Climate Change 2014: Synthesis Report (Cambridge Univ. Press, 2014).
- Mastrandrea, M. D. et al. The IPCC AR5 guidance note on consistent treatment of uncertainties: a common approach across the working groups. Climatic Change108, 675–691 (2011). ArticleGoogle Scholar
- Rogelj, J. et al. Energy system transformations for limiting end-of-century warming to below 1.5 °C. Nature Clim. Change5, 519–527 (2015). ArticleGoogle Scholar
- Statement of the CVF Chair at the UNFCCC COP21 Ministerial Dialogue on the Long-Term Goal (Climate Vulnerable Forum, 2015); http://go.nature.com/29DRiRy
- Rogelj, J. et al. Zero emission targets as long-term global goals for climate protection. Environ. Res. Lett.10, 105007 (2015). ArticleCASGoogle Scholar
- IPCC Climate Change 2014: Mitigation of Climate Change (eds Edenhofer, O. et al.) (Cambridge Univ. Press, 2014)
- Rogelj, J. et al. Differences between carbon budget estimates unravelled. Nature Clim. Change6, 245–252 (2016). ArticleGoogle Scholar
- Meinshausen, M. et al. The RCP greenhouse gas concentrations and their extensions from 1765 to 2300. Climatic Change109, 213–241 (2011). ArticleCASGoogle Scholar
- Fuss, S. et al. Betting on negative emissions. Nature Clim. Change4, 850–853 (2014). ArticleCASGoogle Scholar
- Smith, P. et al. Biophysical and economic limits to negative CO2 emissions. Nature Clim. Change6, 42–50 (2015). ArticleCASGoogle Scholar
- Williamson, P. Scrutinize CO2 removal methods. Nature530, 153–155 (2016). ArticleCASGoogle Scholar
- Obersteiner, M. et al. Managing climate risk. Science294, 786–787 (2001). ArticleCASGoogle Scholar
- Kriegler, E. et al. The role of technology for achieving climate policy objectives: overview of the EMF 27 study on global technology and climate policy strategies. Climatic Change123, 353–367 (2014). ArticleGoogle Scholar
- Lobell, D. B. & Tebaldi, C. Getting caught with our plants down: the risks of a global crop yield slowdown from climate trends in the next two decades. Environ. Res. Lett.9, 074003 (2014). ArticleGoogle Scholar
- Creutzig, F. et al. Bioenergy and climate change mitigation: an assessment. GCB Bioenergy7, 916–944 (2014). ArticleCASGoogle Scholar
- Smith, P. et al. in Climate Change 2014: Mitigation of Climate Change (Edenhofer, O. et al.) Ch. 11 (IPCC, Cambridge Univ Press, 2014). Google Scholar
- Havlik, P. et al. Global land-use implications of first and second generation biofuel targets. Energy Pol.39, 5690–5702 (2011). ArticleGoogle Scholar
- Lotze-Campen, H. et al. Impacts of increased bioenergy demand on global food markets: an AgMIP economic model intercomparison. Agric. Econ.45, 103–116 (2014). ArticleGoogle Scholar
- Riahi, K. et al. Locked into Copenhagen Pledges — Implications of short-term emission targets for the cost and feasibility of long-term climate goals. Technol. Forecast. Soc. Change90A, 8–23 (2013). Google Scholar
- The Emission Gap Report 2015: A UNEP Synthesis Report (UNEP, 2015).
- Rogelj, J., McCollum, D. L., O'Neill, B. C. & Riahi, K. 2020 emissions levels required to limit warming to below 2 °C. Nature Clim. Change3, 405–412 (2013). ArticleCASGoogle Scholar
- Synthesis Report on the Aggregate Effect of the Intended Nationally Determined Contributions FCCC/CP/2015/7 (UNFCCC, 2015).
- Rogelj, J. et al. Paris Agreement climate proposals need a boost to keep warming well below 2 °C. Nature534, 631–639 (2016). ArticleCASGoogle Scholar
- Jaeger, C. C. & Jaeger, J. Three views of two degrees. Reg. Environ. Chang.11, 15–26 (2011). ArticleGoogle Scholar
- Schellnhuber, H. J. Rahmstorf, S. & Winkelmann, R. Why the right climate target was agreed in Paris. Nature Clim. Change6, 649–653 (2016). ArticleGoogle Scholar
- Rogelj, J. & Knutti, R. Geosciences after Paris. Nature Geosci.9, 187–189 (2016). ArticleCASGoogle Scholar
- Mitchell, D. et al. Realizing the impacts of a 1.5 °C warmer world. Nature Clim. Change6, 735–737 (2016). ArticleGoogle Scholar
- James, R. & Washington, R. Changes in African temperature and precipitation associated with degrees of global warming. Climatic Change117, 859–872 (2013). ArticleGoogle Scholar
- Hallegatte, S. et al. Mapping the climate change challenge. Nature Clim. Change6, 663–668 (2016). ArticleGoogle Scholar
- Chadwick, R. & Good, P. Understanding nonlinear tropical precipitation responses to CO2 forcing. Geophys. Res. Lett.40, 4911–4915 (2013). ArticleGoogle Scholar
- Hawkins, E., Joshi, M. & Frame, D. Wetter then drier in some tropical areas. Nature Clim. Change4, 646–647 (2014). ArticleGoogle Scholar
- Bouttes, N., Gregory, J. M. & Lowe, J. A. The reversibility of sea level rise. J. Clim.26, 2502–2513 (2013). ArticleGoogle Scholar
- Schleussner, C.-F., Levermann, A. & Meinshausen, M. Probabilistic projections of the Atlantic overturning. Climatic Change127, 579–586 (2014). ArticleGoogle Scholar
- Drijfhout, S. et al. Catalogue of abrupt shifts in Intergovernmental Panel on Climate Change climate models. Proc. Natl Acad. Sci. USA112, 43 (2015). ArticleCASGoogle Scholar
- Joughin, I., Smith, B. E. & Medley, B. Marine ice sheet collapse potentially underway for the Thwaites Glacier Basin, West Antarctica. Science344, 735–738 (2014). ArticleCASGoogle Scholar
- Rignot, E., Mouginot, J., Morlighem, M., Seroussi, H. & Scheuchl, B. Widespread, rapid grounding line retreat of Pine Island, Thwaites, Smith and Kohler glaciers, West Antarctica from 1992 to 2011. Geophys. Res. Lett.41, 3502–3509 (2014). ArticleGoogle Scholar
- Favier, L. et al. Retreat of Pine Island Glacier controlled by marine ice-sheet instability. Nature Clim. Change4, 117–121 (2014). ArticleGoogle Scholar
- Feldmann, J. & Levermann, A. Collapse of the West Antarctic Ice Sheet after local destabilization of the Amundsen Basin. Proc. Natl Acad. Sci. USA112, 14191–14196 (2015). ArticleCASGoogle Scholar
- Mengel, M. & Levermann, A. Ice plug prevents irreversible discharge from East Antarctica. Nature Clim. Change4, 451–455 (2014). ArticleGoogle Scholar
- Spence, P. et al. Rapid subsurface warming and circulation changes of Antarctic coastal waters by poleward shifting winds. Geophys. Res. Lett.41, 4601–4610 (2014). ArticleGoogle Scholar
- Hellmer, H. H., Kauker, F., Timmermann, R., Determann, J. & Rae, J. Twenty-first-century warming of a large Antarctic ice-shelf cavity by a redirected coastal current. Nature485, 225–228 (2012). ArticleCASGoogle Scholar
- Vuuren, D. P. et al. A new scenario framework for climate change research: scenario matrix architecture. Climatic Change122, 373–386 (2014). ArticleGoogle Scholar
- Steffen, W. et al. Planetary boundaries: guiding human development on a changing planet. Science347, 1259855 (2015). ArticleCASGoogle Scholar
- Lomax, G., Lenton, T. M., Adeosun, A. & Workman, M. Investing in negative emissions. Nature Clim. Change5, 498–500 (2015). ArticleGoogle Scholar
- Meinshausen, M. et al. National post-2020 greenhouse gas targets and diversity-aware leadership. Nature Clim. Change5, 1098–1106 (2015). ArticleGoogle Scholar
- Edenhofer, O. King Coal and the queen of subsidies. Science349, 1286–1287 (2015). ArticleCASGoogle Scholar
- The Coal Gap: Planned Coal-Fired Power Plants Inconsistent with 2 °C and Threaten Achievement of INDCs (Climate Action Tracker, 2015).
- Lelieveld, J., Evans, J. S., Fnais, M., Giannadaki, D. & Pozzer, A. The contribution of outdoor air pollution sources to premature mortality on a global scale. Nature525, 367–71 (2015). ArticleCASGoogle Scholar
- Rogelj, J. et al. Air-pollution emission ranges consistent with the representative concentration pathways. Nature Clim. Change4, 245–252 (2014). ArticleCASGoogle Scholar
- Hulme, M. 1.5 °C and climate research after the Paris Agreement. Nature Clim. Change6, 222–224 (2016). ArticleGoogle Scholar
- INDCs Lower Projected Warming to 2.7 °C: Significant Progress But Still Above 2 °C (Climate Action Tracker, 2015).
Acknowledgements
We acknowledge the work by IAM modellers that contributed to the IPCC AR5 Scenario Database and the World Climate Research Programme's Working Group on Coupled Modelling, which is responsible for CMIP. We thank the climate modelling groups for producing and making available their model output, and the International Institute for Applied System Analysis for hosting the IPCC AR5 Scenario Database. For CMIP, the US Department of Energy's Program for Climate Model Diagnosis and Intercomparison provided coordinating support and led development of software infrastructure in partnership with the Global Organization for Earth System Science Portals. We would like to thank the modelling groups that participated in the Inter-Sectoral Impact Model Intercomparison Project (ISI-MIP) and the Potsdam Institute for Climate Impact Research for hosting the database. The work was supported by the German Federal Ministry for the Environment, Nature Conservation and Nuclear Safety (11_II_093_Global_A_SIDS and LDCs), within the framework of the Leibniz Competition (SAW-2013-PIK-5), from EU FP7 project HELIX (grant no. FP7-603864-2) and by the German Federal Ministry of Education and Research (BMBF; grant no. 01LS1201A1). J.R. received funding from the EU's Horizon 2020 research and innovation programme under grant agreement no. 642147 (CD-LINKS).
Author information
Authors and Affiliations
- Climate Analytics, 10969, Berlin, Germany Carl-Friedrich Schleussner, Michiel Schaeffer, Tabea Lissner & William Hare
- Potsdam Institute for Climate Impact Research, Potsdam, 14473, Germany Carl-Friedrich Schleussner, Tabea Lissner, Anders Levermann, Katja Frieler & William Hare
- Energy Program, International Institute for Applied Systems Analysis, Laxenburg, 2361, Austria Joeri Rogelj
- Institute for Atmospheric and Climate Science, ETH Zurich, Zürich, 8092, Switzerland Joeri Rogelj, Erich M. Fischer & Reto Knutti
- Environmental Systems Analysis Group, Wageningen University and Research Centre, 6708 PB, Wageningen, the Netherlands Michiel Schaeffer
- Woodrow Wilson School of Public and International Affairs, Princeton University, Princeton, 08544, New Jersey, USA Rachel Licker
- Institute of Physics and Astronomy, University of Potsdam, Potsdam, 14476, Germany Anders Levermann
- Lamont-Doherty Earth Observatory, Columbia University, 10964-1000, New York, USA Anders Levermann
- Carl-Friedrich Schleussner